What are the processes Resulting in Analogous genes?
Analogous genes are genes that have identical or similar function but don't share a common ancestor and are therefore unrelated as opposed to homologous genes. The main processes that result in analogous genes or structures are convergent evolution, parallel evolution, and reversals.
Convergent evolution
When two unrelated species over time develop similar structures or genes, we collectively name the processes as convergent evolution. At the genetic level of the hierarchy, we can classify the convergent evolution into independent and collateral genetic evolution.
Independent evolution
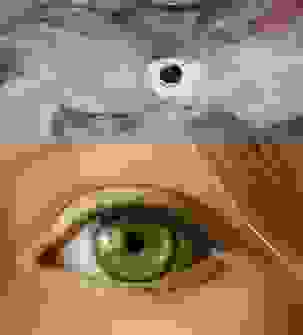
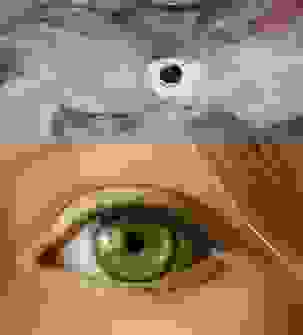
When unrelated mutations occur in separate populations or species, we name the processes as independent evolution.
One famous example of independent convergent evolution that results in analogous structures are bird, insect, and bat wings. Another one is the development of camera-like eyes in cephalopods and vertebrates, such as squid and human eyes (Figure 5). Eyes have independently evolved at least 40 or possibly 65 times.
When humans migrated out of Africa about 60,000-70,000 years ago and populated the northern hemisphere, their skin started to lighten. In the north, there is less sunlight than in Africa. Because light skin allows increased vitamin D synthesis compared to dark skin pigmentation, this was advantageous to the people that made their dwellings in the north.
Collateral evolution
Collateral evolution can occur by (1) a selection of polymorphic alleles that exist in an ancestral population, or by (2) hybridization of genetic material between two divergent populations or species.
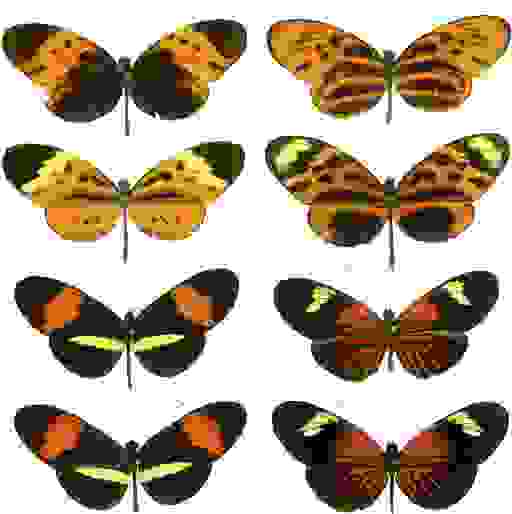
(source: Repeating Patterns of Mimicry. Axel Meyer, PLoS Biology. CC BY 2.5)
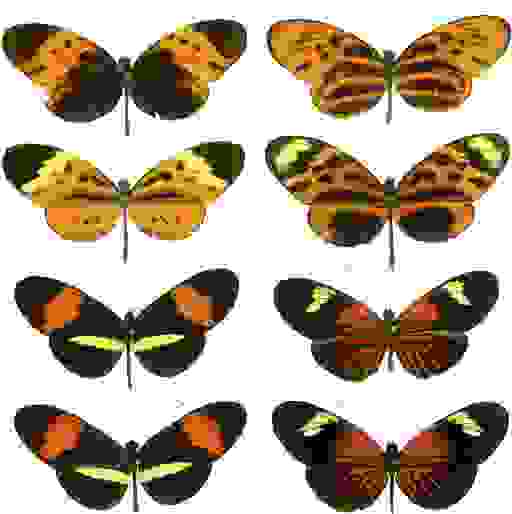
(source: Repeating Patterns of Mimicry. Axel Meyer, PLoS Biology. CC BY 2.5)
(1) By definition, the selection of polymorphic alleles does not result in analogous structures or genes because of the selection pool is present in an ancestral population. Hence, these structures or genes are homologous due to having a common ancestor. Nonetheless, the second category of collateral evolution, hybridization, yields analog genes and structures.
(2) Hybridization is a process of adding genes from one population or species to another. In other words, the gene-flow is not from parent to offspring (Figure 4). We can separate two types of hybridization processes, simple hybridization, and introgression. A simple hybridization results in a hybridization of a single gene or a homogenous set of genes. Introgression results in hybridization of a mixed set of genes and genetic material.
For animal and plant breeding, humans use introgression to improve essential characteristics of animals and plants.
In nature, hybridization is frequently occurring in a wide range of organisms, especially in plants and animals, and even in humans. When hybridization is between species, it results in analogous genetic material.
Butterflies that belong to the genus Heliconius exchange genetic material by hybridizing mimicry associated regions of chromosomes. The genes affect the wing patterning (Figure 6).
Approximately 30-70% of all flowering plant species have hybridization events in their history. Consequently, we can gather that hybridization is an indispensable ingredient for plants to obtain diversity. One natural hybrid is the sunflower, Helianthus anomalus, which is a hybrid of two other sunflowers, H. annuus, and H. petiolaris.
In mammals, hybridization that results in new species is rare. One example is a bat species, Artibeus schwartzi. This bat lives in the Caribbean and is believed to be the result of the hybridization of three other species, A. jamaicensis, A. planirostris, and an unknown extinct bat species.
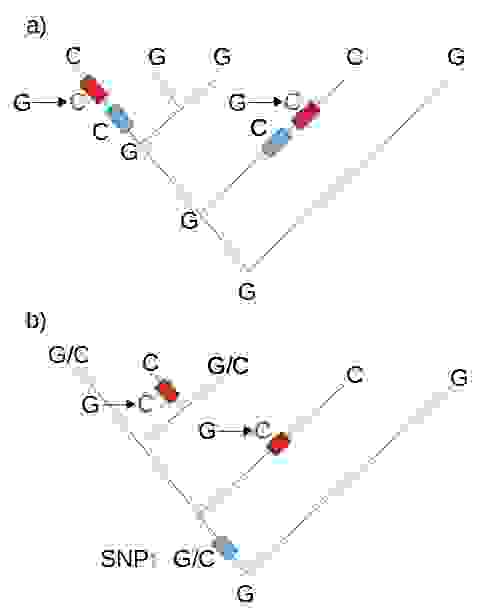
(SNP = single nucleotide polymorphism)
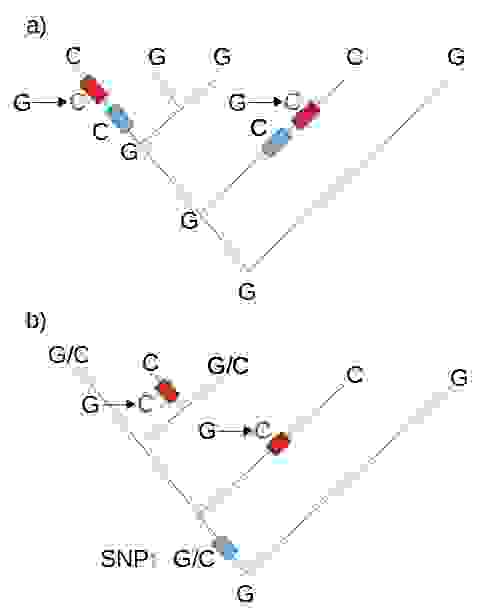
(SNP = single nucleotide polymorphism)
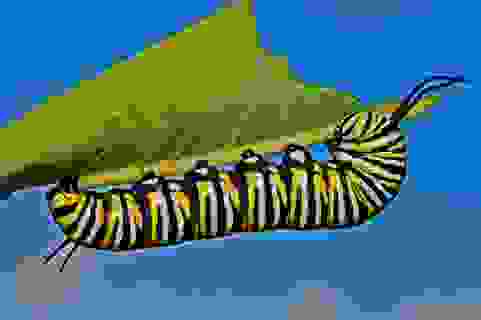
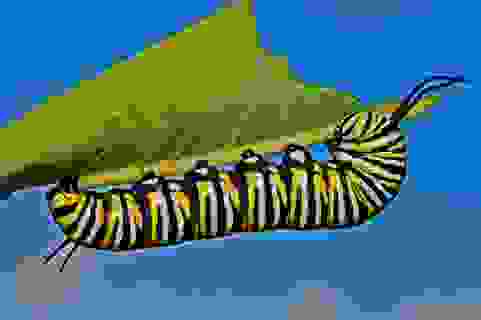
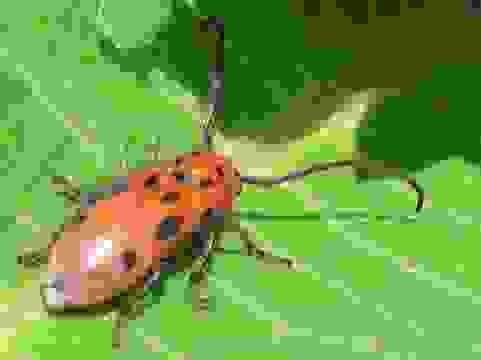
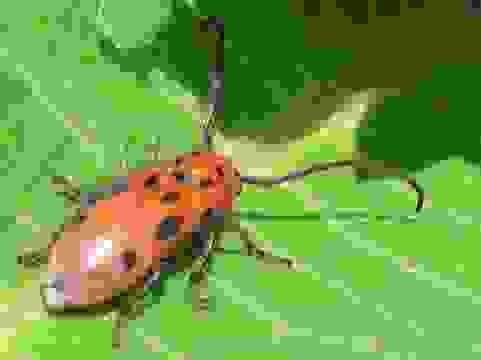
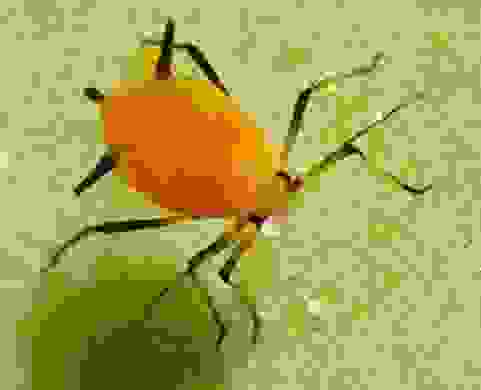
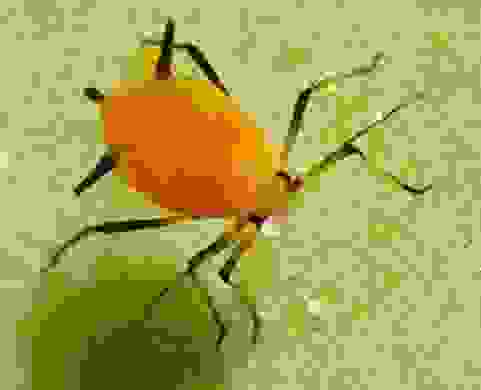
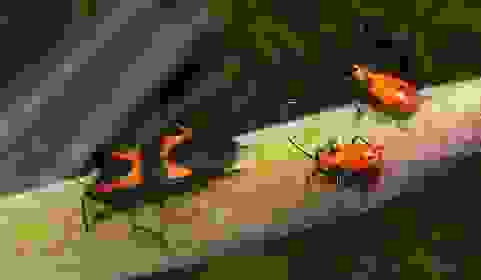
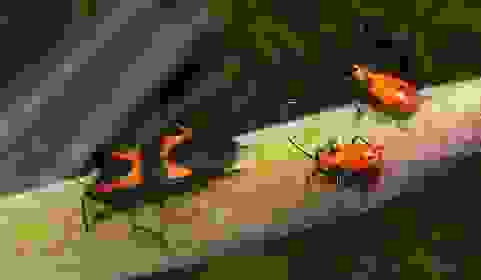
Parallel evolution
In theory, parallel evolution is the development of identical or similar traits in separate species; Thus, the resulting characteristics are analogous.
In practice, parallel evolution is difficult to distinguish from collateral evolution by shared ancestry. One way is to examine the ancestors of each species and see if they share a similar trait. If ancestors lack the feature, we hypothesize that evolution is parallel, otherwise collateral evolution by shared ancestry (Figure 7).
Still, we can question how far back in the evolution we need to look back, and what degree of similarity is acceptable for us to stick with either one of the hypotheses. Further discussion of the point is beyond the scope of this tutorial.
However, only in the parallel evolution process, the result is analogous genes, structures, and traits, and collateral evolution by shared ancestry produces homologs.
An example of parallel evolution is monarch butterfly caterpillar (Danaus plexippus), red milkweed beetle (Tetraopes tetrophthalmus), oleander aphids (Aphis nerii), and large milkweed bug (Oncopeltus fasciatus). All have acquired mutations in parallel in (Na++K+) ATPase genes, allowing them to feed poisonous milkweed plants (Figures 8, 9, 10, and 11).
Read a recent news article in Science: How the monarch butterfly evolved its resistance to toxic milkweed.
· · ·
Reversals - Atavism
Sometimes a trait or a feature that an organism has lost can reappear. Reversals, atavism, therefore, do not produce analogous genes because the gene or genes encoding for the trait has already been present in the organism's genome.
Atavism may be caused by
- 1. a previously suppressed gene becomes active due to a mutation in a regulatory factor that suppressed the expression,
- 2. a variation of time in fetal development by either prolonging or decreasing the development time.
The human embryo initially grows a tailbud and is the most prominent during 32-35 weeks into the pregnancy, but the growth is later switched off (Figure 12). Apoptosis and macrophages will ordinarily remove the tailbud by phagocytosis. However, several reported cases exist where humans also are born with a tail.
The chicken genome contains genes that are involved in tooth development, although birds lost their teeth about 60 to 80 million years ago.
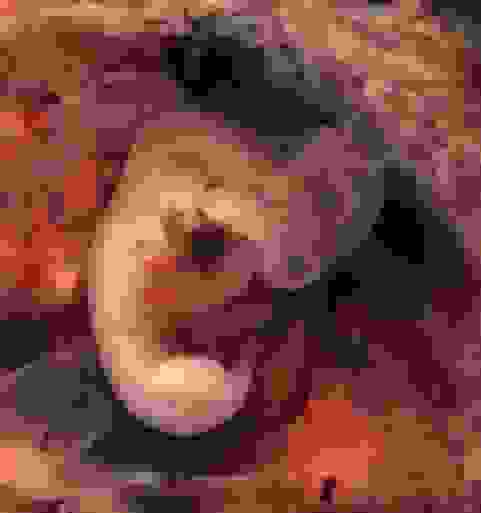
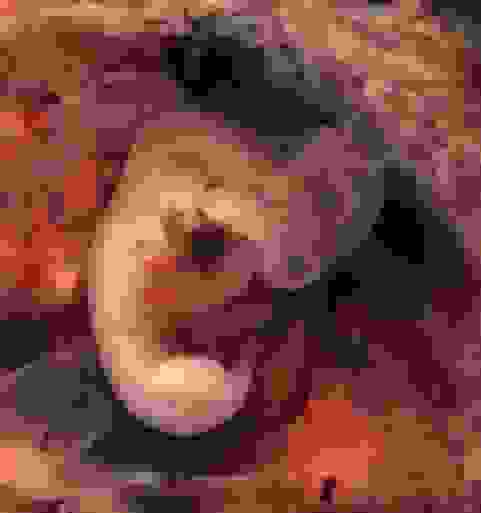
Homology and Levels of Hierarchy
Darwin's theory, descent with modification, explains well the variation of all the different biological structures we can observe in nature. However, throughout history, it has been challenging to define how unique organs and structures arise.
As always, we scientists like to arrange things into distinct categories and concepts. In this case, it was necessary to distinguish between variation due to shared ancestry and novel variation; Thus, the idea of homology was born to refer to shared ancestry among characters.
Historically, structural, functional, and phylogenetic models have been the basis of homology. However, we can observe homology at many different hierarchical levels, and this is where definitions get intricate.
The exact definitions have been often debated, particularly along with the many new insights gained by the use of the latest sequencing technologies.
Depending on the level of the hierarchy, characters and features may or may not be homologous.
One frequently described example is birds' and bats' wings. These forelimbs originate from a common ancestor and are thus homologous structures. On the functional level, however, birds' and bats' wings are not homologous because both species have independently evolved to fly.
The inheritance of DNA and genes are direct copies from parents to offspring; Therefore, the concept of homology is easy to understand at this level. On the other hand, organs and phenotypes don't arise by direct copying but instead involve complex cellular regulatory and extracellular signaling networks.
Homology is not about similarity
Homology is not about similarity. See the above example of Elvis and an impersonator (Figure 1)). Organs that look different can be homologous. For example, a human hand and a bird wing look very dissimilar, but they are both derived from corresponding parts of a common ancestor.
We have arrived at a point where the concept of homology has been under deliberation for the past 150 years. Arising from Richard Owen's original definition of homology in 1843, as "the same organ in different animals under every variety of form and function." Specifically, the meaning of the "same" has regularly been under scrutiny and discussion.
Owen's definition implies descent with modification, associated with phylogenetic and systematic studies. This strategy is historical homology.
Instead of deep-diving into this discussion in detail, we give an overview of the main approaches to how homology can be defined.
On the next page, we introduce concepts, such as GRN Kernel, character identity network, and deep homology.
You may also be interested in the following
Related tutorials
Pair-wise sequence alignment
Pair-wise sequence alignment methods
How to select the right substitution matrix?